In this longer, online-only version of their feature, Professor Gerta Keller et al.* survey the state of mass extinction studies, over 30 years since Walter and Luis Alvarez, Frank Asaro and Helen Michel published their seminal paper on the KT impact layer.
Abstract
Picture - The Deccan Traps of India show layered lava flows forming high (3000m) mountains and covering an area the size of France. Their original extent was about three times the size of France.
The nature and causes of mass extinctions in the geological past have remained topics of intense scientific debate for the past three decades. Central to this debate is the question of whether one or several large bolide impacts, the eruption of large igneous provinces (LIPS), or a combination of the two were the primary mechanism(s) driving the environmental and habitat changes that are universally regarded as the proximate causes for four of the five major extinction events. Recent years have seen a revolution in our understanding of both the interplanetary environment and LIPS eruptions and their environmental effects such that the current impact-kill scenario no longer seems adequate for the KT (KPg) or any other mass extinction. Massive sequential volcanic eruptions and the breakup of giant comets leading to rapid climate change, ocean anoxia and ozone destruction emerge as the leading causes in major mass extinctions.
Keywords: Phanerozoic, mass extinctions, volcanism, impacts, ocean anoxia, climate change
Introduction
Considerable recent research efforts have been directed toward understanding the context and nature of environmental changes that occurred immediately prior to, at and after the five major Phanerozoic mass extinctions. This effort has resulted in significant new data and observations from the fields of geochronology, geochemistry, mineralogy, palaeontology, sedimentology, stratigraphy, palaeomagnetism, volcanology and geophysics. Consequently, the body of scientific evidence based on these new data, and on a large variety of modern analytical techniques, has matured to a point that requires a new critical review of hypotheses concerning the cause(s) of these eco-evolutionary events.
To this end the Natural History Museum, London, hosts an international, multidisciplinary conference (http://www.massextinction.princeton.edu.html; registration at http://www.minersoc.org/mass-extinctions.html) on March 27-29, 2013, that brings together researchers across many disciplines to assess the current records on volcanism, impacts and mass extinctions, and the stratigraphic and geochemical records of environmental change across major mass extinction events. The main goal is to evaluate the respective roles of volcanism, bolide impacts and associated climate and environmental changes in major episodes of species extinctions.
A main goal of this conference is to assess the present status of the historic gap between proponents of different mass extinction cause hypotheses by integrating the varied evidence from different disciplines. The intention is to foster a new, collaborative, interdisciplinary, community-wide approach to resolving outstanding problems in this field. Data and concepts presented and discussed at this conference will have broad implications that will extend both throughout, and well beyond, the geosciences per se because they will summarize the type of important baseline data necessary for understanding ancient and modern species extinctions.
Background
The nature and cause(s) of major mass extinctions in the geological past have remained topics of intense scientific debate for the past three decades. Central to this debate is the question over the roles of one or multiple impacts, the eruption of large igneous provinces (LIP), or a combination of these were the primary mechanism(s) driving the environmental and habitat changes that are universally regarded as the proximate causes for all major extinction events. Along with this scientific discussion there also exists uncertainty over the roles climate, CO2, SO2 and sea-level changes have played in either precipitating or intensifying extinction events.
The most studied major extinction and the most recent occurred 65.5 million years ago at the close of the Cretaceous Period. During this interval the two hypothesized major causal mechanisms, volcanism and impacts, are known to have operated (Fig. 1). Comparable data exists from the other mass extinction intervals, but these data are not as well known or as thoroughly analyzed. Most crucially, recent research on the end-Cretaceous extinction interval has produced many new and unexpected results. These data now need to be integrated into a comprehensive description of this extinction event, and evaluated against the predictions of the various causal hypotheses.
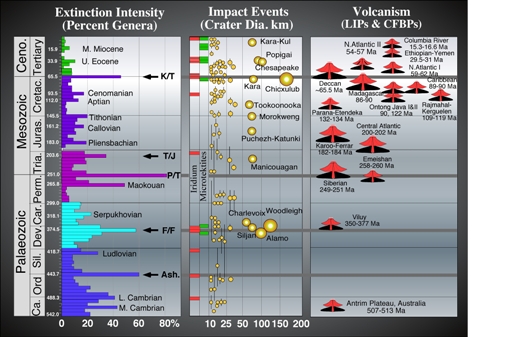
Fig. 1:Mass extinctions, impacts and large igneous provinces during the Phanerozoic. Stratigraphic subdivisions and numerical ages from the 2004 International Stratigraphy Chart (ICS). Genera compilation from Sepkoski (l996), Hallam and Wignall (1997) and MacLeod (2003); impact database from Grieve (1997), LIPS and CFBP database from Courtillot and Renne (2003). Note that the Chicxulub impact predates the K-T boundary by 120,000 years, based on the time scale of Gradstein et al. (2004). (Modified after Keller, 2005)
Despite the attention the ‘dinosaur extinction’ has garnered over the past 30 years in popular and technical scientific publications, we believe it is essential to also go beyond a focus on the end-Cretaceous extinction event to build a general view of the mass extinction phenomenon and its cause(s).
To this end this conference will focus on reviewing the state of information available for all major extinction episodes. Review of the data for the end-Cretaceous event will be especially important in the context of this conference as it serves as a benchmark for understanding the types of research projects that need to be undertaken throughout the geologic column in order to achieve a genuinely comprehensive understanding of the mass extinction phenomena. Here we highlight the major topics of this conference, including the current status, new achievements and outstanding problems. Improvement of our understanding of these events is of outstanding societal importance because of the link that is often drawn between ancient mass extinctions and the current, putatively anthropogenically driven, extinction event.
Volcanic Flood Basalt Eruptions
The largest mass extinctions occurred at the end-Ordovician (~440 Ma), end-Devonian (~360 Ma), end-Permian (~250 Ma), Triassic/Jurassic (~200 Ma), and end-Cretaceous (~65.5 Ma)(Fig. 1). The two main culprits put forward in the early 1980s as potential causes for these extinctions are asteroid impact(s) and volcanic eruptions.
To determine which of these is the likely culprit requires accurate dating of extinctions, impact traces and volcanic remnants. To date only the end-Cretaceous mass extinction is believed to show a direct age link to an asteroid impact (Chicxulub), although some believe the timing is more complex. In contrast, huge basaltic provinces (originally millions of cubic kilometers of lava) have now been shown to have ages corresponding to most mass extinctions with the best accuracy ±1% (Fig. 2).
Fig. 2. Ages of mass extinctions oceanic anoxia events and continental flood basalts. CAMP = Central Atlantic Magmatic Province). Note that only one (end-Ordovician) out of five mass extinctions is not associated with a major continental flood basalt province3.
The most popular case remains that of the Cretaceous-Tertiary (KT) mass extinction, which has both an impact (Chixculub) and flood basalt (the Deccan Traps in India) associated with it. Recent work in India (palaeontology, volcanology and petrology, geochronology and paleomagnetism used jointly) has shown that traces of the impact (Ir anomaly) are sandwiched between lava flows hence one could not have caused the other, a hypothesis that is sometimes made.
Volcanic flows likely occurred as "pulses", with some flows being truly gigantic. Evidence has been found in the field for flows that had original volumes in excess of 10,000 cubic kilometers and erupted in less than a hundred years, possibly only a decade (Fig. 3). For comparison, the largest historical basaltic eruption that occurred in 1783 in Iceland (Laki) erupted some 15 km3 of lava in about a year. So a single huge Deccan flow could have amounted to at least 667 Laki over less than 100 years, or even 100 Laki over about 15 years! The amount of carbon and sulfur dioxides injected in the atmosphere from just one of these very large eruptions would have been truly gigantic, actually on the same order as those ejected by the asteroid impact on Yucatan, which fell on crust rich in carbon and sulfur and vaporized them.
Fig. 3. Relative thickness of Deccan lava flows in each of the three phases of volcanic eruptions calculated as percent of total Deccan trap thickness. Ages based on paleomagnetic time scale4.
Debates on the actual scenarios of the KT extinction are still ongoing but they are likely linked to this enormous pulsed injections of gases into the atmosphere, generating alternating episodes of cooling and warming, shedding acid rain leading to a marine calcification crisis, increasing weathering and terrestrial runoff leading to large nutrient influx into oceans and causing eutrophic or anoxic oceanic conditions hostile to marine life. In the case of the KT mass extinction, the impact would have exacerbated an already heavily stressed environment due to the volcanism, injecting gases in the same amounts and time scales as the individual huge, short volcanic pulses.
Thanks to palaeontology, paleomagnetism and careful dating, we can now identify Deccan Trap eruptions as a series of three phases: phase-1 about 2 m.y. before the KT, phase-2 during the last few hundred thousand years of the Cretaceous and phase-3 in the early Paleocene about three hundred thousand years after the mass extinction (Figure 3). The asteroid impact occurred within phase-2. Each volcanic phase consisted of mega-pulses, each pulse made up of smaller pulses. Current data suggests that the most general cause of mass extinctions has been massive volcanism, even if other events may have intervened and made some of these extinctions even worse.
Oceanic Plateau eruptions and extinctions
Although the potential environmental impact of continental flood basalt provinces has been documented by many authors the possible influence of oceanic plateau eruption on the atmosphere, biosphere and hydrosphere have received much less attention. Oceanic plateau eruptions provide a feasible terrestrial causal mechanism for several second order extinction events. Periods of oceanic environmental crises in the geological record can be identified by the occurrence of black shales, which are indicative of low or oxygen-absent conditions in the deep ocean that correlate with oceanic plateau formation, particularly around the Cenomanian-Turonian boundary (93.5 Ma), in the Aptian (124-112 Ma) and end of Jurassic (~200 Ma) (Figs. 1, 2). Calculated average global surface temperatures around these Cretaceous periods were significantly higher than present and this is most likely due to a marked increase in global atmospheric CO2 content. These phenomena were accompanied by extinction events that resulted in the demise of ~20% of all known genera.
The Cenomanian-Turonian (C-T) and Aptian events have been linked to the formation of oceanic plateaus5,6 . Black shales at the C-T boundary have trace element and radiogenic isotopic signatures consistent with a significant input from oceanic plateau material and this signature coincides with the start of a positive δ13C isotope anomaly. The estimated erupted volume of oceanic plateau lavas is ~1.0 x 107 km3 and may be much higher.
The array of likely effects of oceanic plateau eruptions include plume-related uplift of oceanic lithosphere that may have disrupted transport of cool, oxygenated polar waters into lower latitudes, resulting in increased oceanic anoxia. Release of hydrothermal fluids directly contributed to ocean warming and thus anoxia, since oxygen solubility is considerably reduced in warmer seawater, whereas elevated CO2 levels due to increased volcanic activity leads to higher global temperatures (Fig. 4).
Fig. 4. Diagram summarizing the possible physical and chemical environmental effects of oceanic plateau formation5.
These observed effects of oceanic plateau basalt eruptions support a hypothesis of widespread global oceanic anoxia (and the consequent formation of oil source rocks), which perturbed oceans and atmosphere setting in motion a chain of events that lead to global warming, oceanic anoxia, black shale deposition and ultimately mass extinctions. Alternative potential causes include changes in ocean circulation, accelerated influx of land-derived nutrients through increased weathering and methane hydrate release. Impacts appear unrelated to these oceanic plateau basalt eruptions.
Impacts
That the mass extinction of species at the Cretaceous-Palaeogene boundary was caused by the impact of a 10 km stray asteroid has been a mainstay of both popular journalism and much professional literature since the discovery of a thin layer of iridium at the boundary layer in 1980. As understood at that time, the main reservoir for impactors was the asteroid belt, with a small admixture of risk from comets.
However, recent years have seen a revolution in our understanding of the interplanetary environment and this simple picture no longer seems adequate. Asteroids escape from the main belt through being nudged into 'escape hatches' where orbital resonances with planets remove them, but bodies of 10 km diameter or more are not so easily nudged and the asteroid belt turns out to be deficient by an order of magnitude in this respect. There have probably been 6-18 Chicxulub-type impacts on Earth over the last billion years, as inferred from lunar impact craters, whereas the dynamical studies predict only one from a stray main belt asteroid7.
Another major discovery of recent years is that there are large populations of comets, held in deep freeze, on the fringes of the planetary system. These reservoirs are less collisionally evolved than the main asteroid belt, which has been heavily eroded by mutual impacts, and contain many more large bodies. Comets drift inwards from the reservoirs through the gravitational influence of the giant planets; there is no selection against size. Their delivery to the Earth's environment is a complex problem, which is still under investigation. Most will end up as members of the Jupiter family that is in a flattened system of short-period, Earth-crossing orbits strongly influenced by Jupiter. The first body to be discovered in such reservoirs — Chiron — is about 250 km in diameter (Fig. 5). It is currently in a dynamically unstable region between Saturn and Uranus, and is liable to be thrown into a Jupiter-family orbit on a timescale 0.2 million years8.
Fig. 5. A 2006 Hubble telescope image of Comet P/73 Schwassmann-Wachmann, a small Jupiter family comet, which began to disintegrate in 1995. A Chiron-sized object in this situation, which might have 1 to 10 million times the mass, would constitute a dangerous and prolonged terrestrial hazard.
Comets, when active, are prone to disintegration due to tidal encounters, solar heating and probable internal instabilities, and a temporary sungrazing state is a common phase in the orbital evolution of a Jupiter-family (JF) comet. A Chiron-sized comet may have a mass equivalent to 10,000-15,000 Chixculub-sized impactors, and its progressive disintegration as a JF member would yield a highly enhanced impact hazard at all scales, with a prodigious dust influx into the stratosphere, for the duration of its breakup. The duration of this enhanced hazard is hardly predictable, and could be anywhere from a few thousand to a few hundred thousand years. Galactic disturbances of various sorts may also trigger bombardment through disturbance of the Oort cloud at characteristic intervals of a few tens of millions of years and duration up to a few million years; these will comprise a series of 'spikes' of 0.01-0.1 Myr duration corresponding to the arrival of the largest comets.
Fig. 6. The increasing incompleteness of discovery as one goes into the past is obvious in this age distribution diagram, which is a plot of 40 impact craters with radiometric ages dated to σ≤10 Myr, 35 of them with σ≤5 Myr. The distribution has been smoothed by a rectangular window of width 8 Myr, stepping in 1 Myr intervals. The impression of clustering in time is verified statistically to high confidence level.
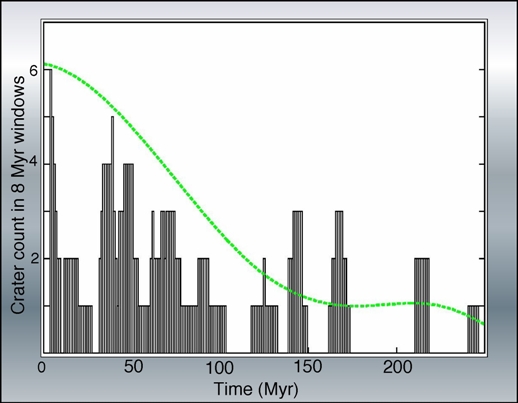
Cratering episodes are manifest in the high-precision impact cratering record of the past 250 Myr
9. Nearly all impact craters >40 km in diameter belong to them, and are accompanied by lesser craters (Fig. 6). The most extreme example is the finding by Jolley and colleagues that the 180 km Chicxulub impact crater in Mexico, and the 24 km Boltysh crater in the Ukraine, were formed within a few thousand years of each other 65 Myr BP. At the level of mass extinction of species, the 10 km solitary asteroid is the least likely of several possible impact scenarios. Theory and observation tell us that at any one time there will be a few bodies in Encke-type orbits, named after the prototype Comet Encke. These orbits are Earth-crossing, with low inclination and periods less than three or four years. Bodies in such orbits are uncoupled from Jovian influence and have dynamical lifetimes of perhaps half a million years before they fall into the Sun, strike a planet or are ejected beyond Jupiter.
Orbital precession ensures that every few thousand years the orbits of Earth and Encke-type body intersect. Every few millions or tens of millions of years one expects a rare giant comet of the type, which one sees on the fringes of the planetary system to cascade all the way down into an Encke-type orbit, where it is at risk of disruption by solar tides. In that situation, the terrestrial impact rate will increase by a factor of some hundreds during the half million years or so of the breakup, with an expectation of say a 10 km body impact somewhere along the route accompanied by many smaller ones, as evidenced by the terrestrial record (Fig. 6). Fireball storms over the duration of the breakup are liable to destroy stratospheric ozone and intermittently reduce incident sunlight; these, as much as impacts, may be major contributors to biological trauma.
Cretaceous-Tertiary (KT) mass extinction
The Cretaceous-Tertiary Boundary (KTB or KPg for Cretaceous-Paleogene) mass extinction is primarily known for the demise of the dinosaurs, the Chicxulub impact as the presumed sole cause and the associated frequently rancorous 30 years old controversy. But there is more to this mass extinction. Among the five major mass extinctions, only the KT can be shown to have a close correspondence between the mass extinction, an iridium anomaly, a large impact crater (Chicxulub) plus one small crater (Boltysh), one of the largest continental flood basalt eruptions (Deccan Traps) and major climate and sea level changes (Fig. 1). Given the revolution in understanding of these events particularly over the past decades this simple impact-kill scenario is simply no longer adequate.
Figure 7. Relative abundance of planktic foraminifera (> 63 µm), stable isotopes, and Ir concentrations in the late Maastrichtian to early Danian Mullinax-1 well, Brazos River, Texas. Note the gradual disappearance of species began during the climate warming and accelerates during the cooling prior to the KT mass extinction. There is no Ir anomaly at the KTB in any of the Brazos River sections, although elevated Ir concentrations are observed at different intervals below and above the KTB associated with redox boundaries10.
The KT mass extinction affected primarily non-avian dinosaurs and marine organisms with calcareous shells (e.g., planktic foraminifera, nannofossils, invertebrates) resulting in an overall 48% decrease in generic diversity (Fig. 1). Many groups died out gradually or decreased in diversity and abundance well before the KT boundary (KTB), including dinosaurs, ammonites, inoceramids and rudists. Only planktic foraminifera, and some
nannofossils, show major, apparently sudden species extinctions coincident with the KTB and Ir anomaly, but even in this group a gradual decline in abundance and diversity of specialized species began with the rapid climate warming about 150 ky before the KTB (Fig. 7).
The most popular hypothesis holds that the Chicxulub impact is the sole cause of the KT mass extinction and to reconcile the age discrepancy the Chicxulub impact ejecta was defined as KTB age11,12. This hypothesis ignores critical aspects of the empirical record, such as the selective nature and variable rates of extinctions, the actual (rather than KT-defined) age of the Chicxulub impact, and Deccan volcanism.
The precise age of the Chicxulub impact is critical because this cause-effect scenario must show a one-to-one correspondence with the KTB mass extinction. This is not the case as can be demonstrated based on the stratigraphic position of the Chixculub impact spherules in several dozen sections through North and Central America, North Atlantic and Caribbean. The oldest impact spherule layer is found in undisturbed marls and claystones of NE Mexico and Texas in the latest Maastrichtian (lower zone CF1, Fig. 8) about 120 ky below the KTB and separated from the impact breccia by a 50 cm limestone in the Chicxulub crater core Yaxcopoil-110,13,14. Through Guatemala, Belize, Haiti, Cuba and deep-sea sites from the Caribbean and North Atlantic impact spherules are generally reworked in early Danian sediments above a major KTB unconformity. This complex pattern of impact spherule distribution is at the core of the decades old controversy over the cause of the KTB mass extinction. Although commonly interpreted as impact-related liquefaction injecting spherule layers into older (and presumably younger) sediments, and an impact-tsunami causing slumps and gravity flows12, this simple one-size-fits-all scenario cannot adequately explain the complex spherule distribution pattern.
Fig. 8. At the Brazos River section in Texas the oldest impact spherule layer is 45-60 cm below two reworked impact spherule layers associated with a sandstone bed. G. Keller pointing to oldest spherule layer now altered to yellow clay, similar to the two green-yellow layers below the sandstone above.
An impact may have been just one of the environmental problems leading up to the KT mass extinction with Deccan volcanism likely the main culprit. Recent investigation of the Deccan main phase-2 in ten deep wells from the Krishna-Godavari Basin revealed four lava megaflows separated by sand, silt and shale with the last megaflow ending at or near the KTB (Fig. 9)15. The biologic response in India was swift and devastating. During Deccan eruptions prior to the first megaflow, planktic foraminifera suffered 50% species extinctions. Survivors suffered another 50% extinctions after the first megaflow leaving just 7 to 8 species. No recovery occurred between the next three megaflows and the mass extinction was complete with the last mega-flow at or near the KTB. The last phase of Deccan volcanism occurred in the early Danian C29n with deposition of another four megaflows accompanied by delayed biotic recovery of marine plankton. Correlative with this intense volcanic phase-2, climate changed from humid/tropical to arid conditions and returned to normal tropical humidity after the last phase of volcanism.
Fig. 9. Composite species ranges of nine wells in the Krishna-Godavari Basin plotted against biostratigraphy and phase-2 and phase-3 lava mega-flows. Cored intervals at the base of the section, below the first phase-2 mega-flow and below the last mega-flow of phase-3 record the most reliable species richness data. A maximum of 28 species in the upper Maastrichtian is typical for middle shelf environments (~100 m depth). Note the mass extinction began with the onset of phase-2 volcanism and ended with the last mega-flow at or near the KTB. Open circles = contamination and reworking, black circles = in situ species15.
The global climatic and biotic effects attributable to Deccan volcanism have yet to be fully investigated. However, preliminary studies from India to Texas reveal extreme climate changes associated with high-stress environmental conditions among planktic foraminifera leading to blooms of the disaster opportunist Guembelitria cretacea during the latest Maastrichtian (Fig. 10). The direct link between Deccan volcanism and the KTB mass extinction is now well documented based on paleontologic, stratigraphic, geochronologic and geochemical evidence.
Fig 10. Blooms of Guembelitria cretacea, a well-known disaster opportunist, mark high stress conditions from India to the eastern Tethys and to Texas correlative with Deccan phase-2 and phase-3 volcanism. These data suggest that Deccan volcanism affected climate, environment and marine biota across the world15.
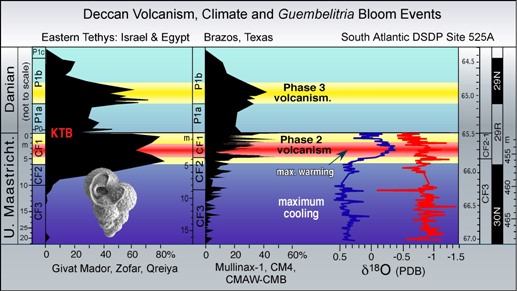
Triassic-Jurassic (TJ) mass extinction
The Triassic period began after the most devastating end-Permian mass extinction and ended with another one, which severely and synchronously affected both the marine and terrestrial ecosystems, coincident with global greenhouse warming and major perturbations in the carbon cycle. The cause for these environmental and biotic calamities has long been puzzling. Widespread acceptance of the Alvarez hypothesis stimulated the search for extraterrestial signatures, but evidence for a putative impact remained unconvincing and inconclusive16. A more viable scenario emerged with U-Pb dating of volcanic ash layers in marine sections that tied the T-J boundary to ~200-201.4 Ma synchronous with the eruptive pulse of the Central Atlantic Magmatic Province (CAMP)17-19. CAMP is one of the largest igneous provinces of the Phanerozoic and heralds the breakup of the supercontinent Pangea. Basaltic lavas erupted over vast areas now divided among four continents (North and South America, Africa and Europe). This magmatic province is now widely thought to have triggered the cascade of linked biotic and environmental catastrophes in Earth’s system.
The TJ catastrophe caused the extinction of conodonts and near-extinction of ammonoids with possibly a single evolutionary lineage surviving. The flourishing reef communities disappeared, leaving a prominent earliest Jurassic gap (Fig. 11). Terrestrial life also suffered a crisis, which is best known from macroflora preserved in Greenland and the pollen record elsewhere. Dinosaurs were the winners benefiting from the ecologic opportunity granted by the high extinction rate of the dominant crurotarsan reptiles at the TJ boundary.
Fig. 11. Synthesis of the TJ catastrophe showing changes in the carbon cycle, increased CO2, rising sea level, ocean anoxia and changes in the marine biota20.
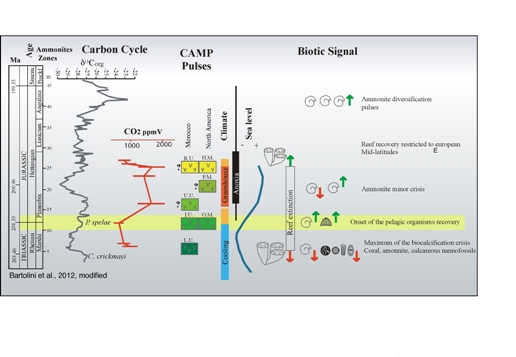
Atmospheric carbon dioxide concentrations abruptly increased along with extreme greenhouse warming as inferred from the lower density of stomata on fossil Ginko leaves. Hot and humid conditions are also suggested by an abundance of kaolinite among clay minerals in TJ boundary sections21. Negative carbon isotope excursions accompany these TJ boundary events. Although the number of pulses and the magnitude and timing of these excursions are still debated, modeling studies suggest that neither mantle-derived volcanic outgassing nor depressed primary biological productivity can adequately explain these observations. The addition of isotopically light carbon is conceivable from dissociation of gas hydrates that store bacterially produced methane in marine sediments, or the release of thermogenic methane where ascending CAMP lavas came into contact with coal deposits. In either case, CAMP magmatism is likely the trigger, with the effects of initial volcanic CO2-driven warming greatly amplified through injection of methane resulting in drastic changes in the atmospheric greenhouse gas budget. Analysis of cyclic sedimentary rocks across the TJ boundary suggests that episodes of carbon cycle perturbations were short-lived events, lasting only a few tens of thousands of years.
Whereas extreme global warming is held responsible for the mass extinction by many researchers, others argue that actually short pulses of cooling, induced by volcanically derived sulphate aerosols, may have been equally detrimental to the ecosystems. Although kill mechanisms and details of the chain of events continue to be debated, the case for linking CAMP volcanism to environmental and biotic change at the TJ boundary now appears well-founded on multiple lines of stratigraphic, geochronologic, geochemical and paleontological evidence.
Fig. 12. Faunal turnover, impacts and volcanism across the Permian-Triassic transition5.
The End-Permian (PT) mass extinction
The end-Permian mass extinction event (252 million years ago) marks the most severe crisis of the fossil record, culminating in a spectacular coup de grâce at the end of the Permian (Fig. 12). In the oceans the extinction losses were devastating and remarkably non-selective. Virtually all species in reef ecosystems dominated by calcareous sponges and algae, and most species dominating tropical carbonate platforms (brachiopods, corals, forams) went extinct along with major extinctions in organisms with siliceous skeletons (sponges and radiolaria). The soft-bodied organisms of the oceans, such as the worms and sea cucumbers, have a poor fossil record but they leave burrows (trace fossils), such as the complex swirly marks of Zoophycus, which disappeared in the Early Triassic (Fig. 13). This suggests equally devastating losses amongst the soft-bodied fauna.
On land extinctions were as catastrophic as those in the oceans. Many insect groups disappeared as well as large predators (gorgonopsids) and large herbivores (pareiasaurs). Terrestrial plant communities probably suffered the worst crisis in Earth history. Gymnosperm trees dominated forests at this time, with Glossopteris being the most famous representative from the high latitude southern forest whilst Gigantopteris was its equatorial equivalent. The end-Permian saw the loss of these forests and the “extinction” of coal formation: no coals formed in any location for the next 15-20 million years. The immediate aftermath of the extinction saw the proliferation of fungal or algal spores and pollen of still questionable affinities. These were soon replaced by Lycophytes (club mosses), which dominated the forests of the Carboniferous and their re-emergence in the early Triassic led to a strangely old-fashioned style terrestrial ecosystem.
What caused this mass extinction? Attempts at linking it a meteorite impact have been unsuccessful. The Siberian Traps, a vast area of flood basalts that erupted at precisely the time of the crisis, appears to be the smoking gun. However, it is one thing to highlight a coincidence and another to try and link these two very different geological phenomena. Volcanic eruptions emit a lot of gases of which carbon dioxide and sulphur dioxide are the most voluminous.
Fig. 13. Burrowing traces of Zoophycos. Their disappearance at the PT extinction indicates that these organisms were also victims of this mass extinction.
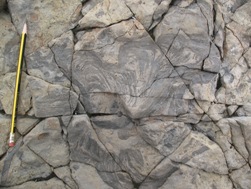
Their effects on the world’s climate are fundamentally different; the former is a greenhouse gas with a legacy that lasts thousands of years whilst the latter causes cooling for just a few years before raining out as acid rain. Gas release from Siberian magma eruptions alone would not have been lethal, but the addition of gases released from coal and evaporates baked by the erupting magma could have done the deed. The recent discovery of fossil fly ash (indicative of subterranean burning of coal beneath lava) in latest Permian sediments from the Arctic provides evidence that this process may have vastly increased CO
2 emissions.
Even as we begin to understand the nature of giant volcanism like the Siberian Traps we are still a long way of knowing how the gas emissions cause environmental damage sufficiently severe to trigger a catastrophe of the size seen at the end of the Permian. Geological evidence indicates the crisis was associated with warming and the spread of anoxia in marine waters whilst the possibility of ocean acidification has recently been added to potential woes of marine life at this time. On land the killing mechanism remains more enigmatic. Sulphur dioxide emissions can cause short-term cooling, likely a factor in the northern hemisphere. The global devastation of plant communities points to atmospheric problems such as ozone depletion. If correct, this suggests that Siberian volcanism also released significant volumes of ozone-destroying halogen compounds as well as more familiar volcanic gases: a truly noxious cocktail.
End-Devonian mass extinction
The Late Devonian mass extinction (Frasnian-Famennian (FF) boundary dated at 376 Ma) occurred over several million years with a series of extinction pulses in the latest Frasnian. Major reef builders (corals and stromtoporoid) were the most severely affected. Among other marine invertebrates 70% of the taxa did not survive including trilobites, brachiopds, ammonites and bryozoans but also conodonts, jawless fish and placoderms. Terrestrial environments were relatively little affected.
Extraterrestrial impacts, oceanographic and climatic changes have been proposed as causes for this mass extinction and are still debated. A possible impact close to the FF mass extinction is the 65-75 km diameter Siljan Ring crater of Sweden (377-378 Ma) (Fig. 14). However, this relatively small, single impact cannot explain the series of extinction pulses in the latest Frasnian and although multiple impacts have been hypothesized there is no evidence to date22,23. Moreover, Os isotope data show no evidence of a major meteoric input in the latest Frasnian24.
The role of volcanism and its potential influence on Late Devonian climate are currently not well constrained. The Viluy Traps in Eastern Siberia were suggested as potentially coincident with the Late Devonian mass extinction. However, preliminary dating suggests an age of around 370 Ma25 and thus a 3-4 m.y. younger age than the mass extinctions.
Fig. 14. Carbon isotopes, sea surface temperature (SST), sea level and extinction records for the Late Devonian mass extinction. Kellwasser horizons correspond to anoxic episodes in the World’s oceans. Carbon and SST records shown for two different sections. SST reconstructed from oxygen isotopes of biogenic apatite.
Changes in the oceanographic and climatic system are relatively well documented for this critical interval. Most prominent are the black shale facies that developed in the world’s ocean during the latest Frasnian (Lower Kellwasser event) and at the Frasnian-Famennian boundary (Upper Kellwasser event) and mark anoxic to euxinic conditions based on trace element geochemistry, pyrite framboid size populations and organic biomarkers indicating photic zone anoxia26. Anoxic conditions were probably the result of higher primary productivity triggered by an intensified input of nutrients to the oceans. Several events contributed to increased nutrient influx and eutrophication of the oceans, including enhanced weathering due to uplift during the Eovariscan orogeny, the evolution of land plants and phosphorus from volcanic ashes.
Enhanced burial of organic carbon is documented by positive carbon isotope excursions of inorganic and organic carbon during the anoxic episodes (Fig. 14) leading to a drawdown of atmospheric and oceanic CO2 levels and culminating in significant climate cooling. These short-term cooling episodes were superimposed on longer term climate warming beginning in the middle Devonian with up to 8°C warmer temperatures reached in the latest Frasnian to early Famennian27. Current knowledge thus suggests that recurrent eutrophication, anoxia, global carbon cycle perturbations and climate changes may have had a severe impact on faunal diversity in the Late Devonian.
End-Ordovician mass extinction
The end-Ordovician marks a rapid rise in extinctions concomitant with a rise in originations, which ended the Great Ordovician Biodiversification Event (GOBE) and established the Palaeozoic biodiversity plateau that persisted until the end of the Palaeozoic Era. In the marine realm about 20% families, 40% genera and 85% species went extinct.
The mass extinction comprised two extinction pulses (Fig. 15). The first pulse occurred at, or just below the base of the Hirnantian Stage (Normalograptus extraordinarius graptolite Zone) and targeted benthic organisms in deep and shallow-water environments, but less at mid-water depths, and graptolites and nektonic groups among the plankton. Major suggested killing mechanisms include glacially-induced cooling, falling sea level and chemical recycling in the oceans, but a general consensus is lacking.
Fig. 15. The two Late Ordovician (Hirnantian) mass extinction are associated with glaciation31 and geochemical proxies32-33. These data support the relative brevity of the event, dramatic changes in ocean productivity and widespread cooling during the Hirnantian
The second extinction pulse occurred in the middle Hirnantian Stage (N. persculptus graptolite Zone) and is associated with a strong sea level rise and ocean anoxia marking a brief warm event (Fig. 15). Extinctions were less selective, wiping out faunas across the range of water depths and killing off mainly survivors from the first extinction pulse28-29. The extinctions dramatically reduced the number of biogeographical provinces from ten provinces to nine leaving only five in the Early to Middle Silurian.
Despite our increasing knowledge of Ordovician climate there is no consensus as to how climate change directly influenced global biodiversity30. Today temperature is an important factor in the distribution of organisms, defining biogeographical provinces that follow the climate belts in the marine realm. Palaeobiogeographical studies of pelagic organisms through the Late Ordovician indicate that during the Hirnantian glaciation the latitudinal temperature gradient steepened, organisms migrated towards the equator, and extinction was highest in the sub-polar climate zone, which contracted along with its habitats. Two other mechanisms have also been invoked to restrict or destroy habitats during the mass extinction. Firstly, a major sea level fall (~100m) during the build-up of the Hirnantian ice sheets reduced shelf area and increased the pressure on habitable space in the outer shelf. Secondly, the ongoing amalgamation of microcontinents during the Caledonian Orogeny would also have reduced shelf area.
Glaciation and likely positive feedbacks during the Hirnantian are sufficient to provide an explanation for Hirnantian mass extinction, within the Earth surface system. The major readjustments that took place within communities under the influence of a rapidly changing climate provide lessons for the future as anthropogenic warming appears to be initiating an Anthropocene mass extinction.
Conclusions
Thanks to paleontology, sedimentology, geochemistry and careful dating, the record for all five mass extinctions appears clear. None of them show a single instantaneous event associated with the mass extinction and causing sudden environmental collapse. All of them reveal prolonged periods of high stress before and after mass extinctions and three show multiple extinction phases (PT, end-Devonian, end-Ordovician), sometimes separated by hundreds of thousands of years. Extinction patterns are varied, sometimes affecting marine life more than terrestrial life, but they are invariably selective and predominantly target shelly critters that depend on secretion of calcium carbonate.
Despite these common patterns, no single cause has emerged, suggesting that multiple causes may produce the same catastrophic environmental effects. Current data suggests the leading cause is massive volcanism (large igneous provinces), which is associated with four out of the five mass extinctions. Impacts, particularly the breakup of large comets could produce prolonged environmental destruction in much the same way as volcanism, but this still has to be investigated. Should the two coincide, as during the KT mass extinction, this would almost certainly cause a major mass extinction as one would exacerbate the other injecting huge amounts of carbon and sulfur dioxides and other gases into the atmosphere, causing acid rain and ocean acidification, fireball storms, destruction of the ozone and climate changes. Some or all of these effects are observed or speculated to have occurred during all five mass extinctions. However, at least one mass extinction event (end-Ordovician) cannot be associated with impacts or volcanism, but shows major climate changes apparently leading to ocean anoxia and two extinction phases.
References
- Gradstein F., Ogg J., Smith A. 2004. A Geologic Time Scale, Cambridge, UK,Cambridge University Press, 598 pp.
- Keller G. 2005. Impacts, volcanism and mass extinctions: random coincidence or cause & effect? Australian Journal of Earth Sciences 52: 725-757
- Courtillot V.C. 2009. Nouveau Voyage au Centre de la Terre, Odile Jacob, Paris, France, 154pp.
- Chenet A.-L., Quidelleur X., Fluteau F., Courtillot V. 2007. 40K/40Ar dating of the main Deccan large igneous province: further evidence of KTB age and short duration. Earth and Planetary Science Letters 263: 1-15.
- Kerr A.C. 2005. Oceanic LIPs: The kiss of death. Elements 1: 289-292.
- Kuroda J., Tanimizu M., Hori R.S., Suzuki K., Ogawa, N.O., Tejada M.L.G., Coffin M.F., Coccioni R., Erba E., Ohkouchi N. 2011. Lead isotopic record of Barremian-Aptian marine sediments: implications for large igneous provinces and the Aptian climatic crisis. Earth and Planetary Science Letters 307: 126-134.
- Minton D.A., Malhotra R. 2010. Dynamical erosion of the asteroid belt and implications for large impacts in the inner Solar System. Icarus 207: 744 – 757.
- Hahn G., Bailey M.E. 1990. Rapid dynamical evolution of giant comet Chiron. Nature 348: 132 – 136.
- Napier W.M. 2006. Evidence for cometary bombardment episodes. Monthly Notices of the Royal Astronomical Society 366: 977-982.
- Keller G. 2011. KT Mass Extinction in marginal and open marine environments: Texas and Tunisia. In: Keller G. & Adatte T. (eds.) SEPM Special Publication 100: 197-226.
- Molina E., Alegret L., Arenillas I., Arz J. A., Gallala N., Hardenbol J., von Salis K., Steurbraut E., Vandenberghe N., Zaghbib-Turki D. 2006. The Global boundary Stratotype Section and Point for the base of the Danian stage at El Kef, Tunisia - original definition and revision. Episodes 29: 263-273.
- Schulte P. and 40 others, 2010. The Chicxulub asteroid impact and mass extinction at the Cretaceous-Paleogene boundary. Science 327: 1214-1218.
- Keller G., Adatte T., Berner Z., Pardo A., Lopez-Oliva L. 2009. Age and biotic effects of the Chicxulub impact in Mexico. J. Geological Society of London 166: 393-411.
- Keller G., Adatte T., Stinnesbeck W., Rebolledo-Vieyra M., Urrutia Fuccugauchi J., Kramar G., Stueben D. 2004. Chicxulub predates the K/T boundary mass extinction. National Academy of Sciences (USA) Proceedings 101: 3753-3758.
- Keller G., Adatte, T., Bhowmick P.K., Upadhyay H., Dave A., Reddy A.N., Jaiprakash B.C. 2012. Nature and timing of extinctions in Cretaceous-Tertiary planktic foraminifera preserved in Deccan intertrappean sediments of the Krishna-Godavari Basin, India. Earth and Planetary Science Letters 341-344: 211-221.
- Olssen P.E., Kent D.V., Sues H-D., Koeberl C., Huber H., Montanari A., Rainforth E.C., Fowell S.J., Szajna M.J., Hartline B.W. 2002. Ascent of dinosaurs linked to an iridium anomaly at the Triassic-Jurassic boundary. Science 296: 1305-1307.
- Pálfy J., Mortensen J.K., Carter E.S., Smith P.L., Friedman R.M., Tipper H.W. 2000. Timing the end-Triassic mass extinction: First on land, then in the sea? Geology 28: 39-42.
- Schaltegger U., Guex J., Bartolini A., Schoene B., Ovtcharova M. 2008. Precise U-Pb age constraints for end-Triassic mass extinction, its correlation to volcanism and Hettangian post-extinction recovery. Earth and Planetary Science Letters 267: 266-275.
- Schoene B, Guex J, Bartolini A, Schaltegger U., Blackburn T.J. 2010. Correlating the end-Triassic mass extinction and flood basalt volcanism at the 100 ka level. Geology 38: 387-390.
- Bartolini A., Guex J., Spangenberg J., Taylor D. Schoene B., Schaltegger U., Atudorei V. 2012. Disentangling the Hettangian carbon isotope record: implications for the aftrmath of the end-Triassic mass extinction. Geochemistry, Geophysicss and Geosystems 13: 1-11.
- Pálfy J., Zajzon N. 2012. Environmental changes across the Triassic-Jurassic boundary and coeval volcanism inferred from elemental geochemistry and mineralogy in the Kendlbachgraben section (Northern Calcareous Alps, Austria). Earth and Planetary Science Letters 335: 121-134.
- McGhee G.R. 2001. The “multiple impacts hypothesis” for mass extinction: a comparison of the Late Devonian and the late Eocene. Palaeogeogr. Palaeoclimatol. Palaeoecol. 176: 47–58.
- Sandberg C.A., Morrow J.R., Ziegler W. 2002. Late Devonian sea-level changes, catastrophic events, and mass extinctions. In Koeberl C., MacLeod K.G. (eds), Catastrophic Events and Mass Extinctions: Impacts and Beyond, Geol. Soc. Ameria Special Paper 356: 473–487.
- Gordon G.W., Rockman M., Turekian K.K., Over J. 2009. Osmium isotopic evidence against an impact at the Frasnian–Famennian boundary. American Journal of Science 309: 420–430.
- Courtillot V., Kravchinsky V.A., Quidelleur X., Renne P.R., Gladkochub F. 2010. Preliminary dating oft he Viluy traps (Eastern Siberia): Eruption at the time of Late Devonian extinction events? Earth and Planetary Science Letters 300: 239-245.
- Marynowski L., Rakocinski M., Borcuch E., Kremer B., Schubert B.A., Jahren A.H. 2011. Molecular and petrographic indicators of redox conditions and bacterial communities after the F/F mass extinction (Kowala, Holy Cross Mountains, Poland). Palaeogeography, Palaeoclimatology, Palaeoecolology 306: 1-14.
- Joachimski M.M., Breisig S., Buggisch W., Talent J.A., Mawson R., Gereke M., Morrow J.M., Day J., Weddige K. 2009. Devonian climate and reef evolution: insights from oxygen isotopes in apatite. Earth and Planetary Science Letters 284: 599-609.
- Rasmussen C.M.Ø., Harper D.A.T. 2011a. Did the amalgamation of continents drive the end ordovician mass extinctions? Palaeogeography, Palaeoclimatology, Palaeoecology 311: 48-62.
- Rasmussen C.M.Ø., Harper D.A.T. 2011b. Interrogation of distributional data for the end-Ordovician crisis interval: where did the disaster strike? Geological Journal 46: 478-500.
- Armstrong H. A. 2007. On the cause of the Ordovician glaciation. In Williams, M., Haywood, A. & Gregory, J. (eds.), Deep time perspectives on climate change, Special Publication of the Geological Society of London, the Micropalaeontological Society and Geological Society of London, p. 101-121.
- Finnegan S., Bergmann K., Eiler J.M., Jones D.S., Fike D.A., Eisenman L., Hughes N.C., Tipati A.K., Fischer W.W. 2011. The magnitude and duration of the Late Ordovician-Early Silurian glaciation. Science 331: 903-906.
- LaPorte D.F., L., Holmden C., Patterson W. P., Loxton J. D., Melchin M. J., Mitchell C. E., Finney S. C., Sheets H. D. 2009. Local and global perspectives on carbon and nitrogen cycling during the Hirnantian glaciation. Palaeogeography, Palaeoclimatology, Palaeoecology 276: 182-195.
- Hammarlund E.U., Dahl T.W., Harper D.A.T., Bond D.P.G., Nielsen A.T., Bjerrum C.J., Schovsbo N.H., Schönlaub H.P., Zalasiewicz J.A., Canfield D.E. 2012. A sulfidic driver for the end-Ordovician mass extinction. Earth and Planetary Science Letters 331–332: 128-139.
Authors
Gerta Keller1, Howard Armstrong2, Vincent Courtillot3, David Harper2, Michael Joachimski4, Andrew Kerr5, Norman MacLeod6, William Napier7, József Palfy8, Paul Wignall9
1: Department of Geosciences, Princeton University, Princeton NJ 08540, USA; 2: Department of Earth Sciences, Durham University, South Road, Durham DH1 3LE, UK; 3: Equipe de Paléomagnétisme, Institut de Physique du Globe, UMR 7154, et Science de la Terre de l’Environment et des Planètes, Université Paris Diderot, Sorbonne Paris, France; 4: GeoZentrum Nordbayern, University of Erlangen-Nuremberg, 91054 Erlangen, Germany; 5: School of Earth and Ocean Sciences, Cardiff University, Cardiff, Wales, UK CF10 3AT; 6: Earth Sciences Department, The Natural History Museum, Cromwell Road, London, SW7 5BD; 7: Buckingham Centre for Astrobiology, University of Buckingham, Buckingham MK18 1EG UK; 8: Dept. of Physical and Applied Geology, Eötvös University, Budapest, H-1117 Hungary; 9: School of Earth and Environment, University of Leeds, LS2 9JT, UK.